Chapter 1 Introduction
1.1 Research Background
Crystal orientation technology is a critical branch of crystallography, widely applied in modern scientific research and industrial production. In the laser industry, crystal orientation directly influences the output performance and stability of lasers. In the field of optical device manufacturing, crystal orientation optimizes the performance of optical components such as lenses and windows. In materials science, orientation studies provide essential technical support for the development of functional materials and novel composite materials.
With advancements in technology, crystal orientation techniques have evolved from traditional manual operations to high-precision measurements relying on advanced instruments. During this progression, optical methods, mechanical methods, and modern instrumentation technologies—such as laser interferometers and electron microscopes—have emerged as mainstream solutions, addressing the orientation needs of various crystal types. Concurrently, research on crystal orientation has propelled advancements in X-ray diffraction theory and nanotechnology, offering powerful tools for scientific experimentation.
Currently, with the continuous development of automation and intelligent technologies, crystal orientation technology is advancing toward greater efficiency and precision. In the future, research on crystal orientation at the nanoscale and the application of multifunctional composite materials will become key focuses in the field. This not only introduces new technical demands for industrial production but also presents fresh challenges and opportunities for academic research.
Chapter 2 Theoretical Foundations of Crystal Orientation
2.1 Crystal Structure and Symmetry
The indispensability of crystals in modern science and industry stems fundamentally from the precise arrangement of their internal atoms. This arrangement forms a lattice structure, and the geometric characteristics of the lattice determine the crystal’s symmetry. The symmetries we commonly refer to—including translational symmetry, rotational symmetry, mirror symmetry, and inversion symmetry—are not merely mathematical concepts but the very origin of a crystal’s physical properties.
In the field of crystal orientation, the Bravais lattice provides a unique perspective. By categorizing the spatial structures of crystals into 14 fundamental types, we can clearly describe the geometric arrangement of crystal lattices. This classification method reveals the spatial symmetry of crystals and helps us understand why certain crystals exhibit distinctive physical and optical properties. For example, the cubic crystal system is renowned for its high symmetry, which endows crystals with uniform mechanical and optical characteristics, making them particularly suitable for applications in laser crystals and optical device manufacturing.
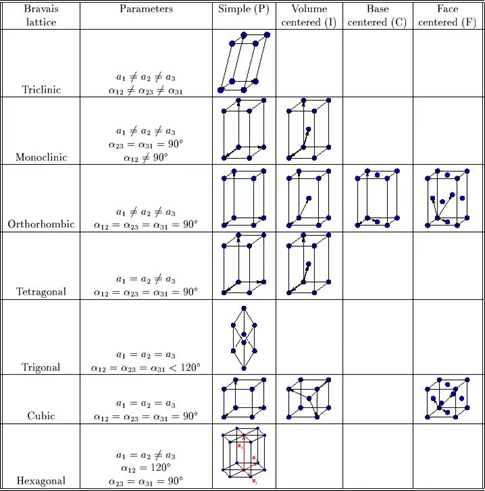
Bravais lattice
Notably, crystal symmetry is inextricably linked to orientation techniques. The goal of orientation is to expose or utilize a specific crystallographic direction, and understanding symmetry is the first step. Without precise knowledge of symmetry, subsequent optical or mechanical methods cannot achieve optimal results.
In summary, crystal symmetry is not only a focal point of theoretical research but also the practical foundation guiding orientation technology. Through the analysis of symmetry features, we can perform crystal orientation more efficiently and accurately, providing robust support for scientific research and industrial applications.
2.2 Miller Indices and Crystallographic Plane Representation
In crystal orientation, the representation of crystallographic planes is a critical issue because the relative position of crystal planes to the axes directly determines the optical, electrical, and mechanical properties of the crystal. As a mathematical language, Miller Indices provide a precise and standardized method to describe the position and orientation of crystal planes.
Miller Indices are represented by three integers, which are the smallest whole-number multiples of the reciprocals of the plane’s intercepts with the crystallographic axes. Specifically, the reciprocals of the intercepts of the plane with the axes are taken and then reduced to the smallest integers. For example, the Miller Indices (hkl) describe the plane’s intersection relationship with the three crystallographic axes. This method standardizes the representation of crystal planes, avoiding confusion between different descriptive approaches.
In practical applications, Miller Indices are not merely theoretical tools but also play a vital guiding role in crystal cutting, polishing, and growth. For instance, in the production of laser crystals, specific crystallographic orientations influence the efficiency and stability of laser output. By using Miller Indices, the desired crystal planes for processing can be precisely determined, ensuring the final product meets design specifications.
More importantly, Miller Indices also enable the automation and intelligence of crystal orientation. In modern instrumentation, Miller Indices are often combined with X-ray diffraction techniques to automatically identify crystallographic orientations. This integration significantly improves measurement efficiency and lays the foundation for high-precision crystal orientation.
2.3 Bragg’s Law and Diffraction Theory
In crystal orientation technology, Bragg’s Law is a fundamental theoretical principle that provides a direct and effective tool for understanding the internal structure and orientation of crystal planes. Bragg’s Law reveals the diffraction patterns of X-rays interacting with crystals and establishes a simple mathematical relationship between interplanar spacing and the angle of incidence, offering a scientific basis for crystal orientation operations.
The core equation of Bragg’s Law is:
nλ = 2d sinθ
- n is the order of diffraction,
- λ is the wavelength of the incident X-rays,
- d is the interplanar spacing, and
- θ is the angle of incidence.
This equation states that when X-rays strike a crystal at a specific angle, the reflected waves from different crystal planes undergo constructive interference, producing diffraction peaks. By analyzing the positions and intensities of these peaks, the structural information of the crystal can be deduced.
Bragg’s Law is not only foundational for theoretical research but also an essential tool in practical applications. In crystal orientation, X-ray diffraction (XRD) is widely used to determine the orientation of crystal planes. For example, during the processing of laser crystals, XRD can accurately locate the optical axis direction, ensuring optimal laser performance. When combined with Miller Indices, the calculation of crystallographic orientations becomes more efficient, providing technical support for large-scale production needs.
Furthermore, the applications of Bragg’s Law in modern science and technology continue to expand. With the widespread use of high-resolution X-ray equipment, studies on the microstructure of crystals have become more detailed. For instance, multi-axis diffraction measurements can reveal internal stress distributions in crystals, thereby optimizing their mechanical properties. These advancements not only enhance the precision of crystal orientation but also open new possibilities for the research and development of novel materials.
Chapter 3 Technical Methods of Crystal Orientation
3.1 Optical Methods
Optical methods represent one of the most traditional yet widely used techniques in crystal orientation, primarily including the Laue Method and X-ray single-crystal diffraction. These approaches determine crystallographic plane or axis orientations by analyzing the interaction between light/X-rays and crystals, offering advantages such as simplicity and non-destructiveness.
The Laue Method, one of the earliest optical techniques for crystal orientation, employs polychromatic X-rays incident on a crystal to analyze diffraction patterns formed by reflected or transmitted beams. This method reveals the crystal’s symmetry and orientation and is commonly applied for rapid symmetry assessment and preliminary orientation—for instance, during intermediate stages of crystal growth to evaluate structural quality.
In contrast, X-ray single-crystal diffraction is renowned for its high precision, making it ideal for scenarios requiring exact crystallographic plane determination. By adjusting the X-ray incidence angle and recording diffraction peak positions/intensities, researchers can reconstruct a crystal’s three-dimensional structure. This technique is indispensable in scientific research, such as exploring novel material structures or optimizing functional crystal orientations.
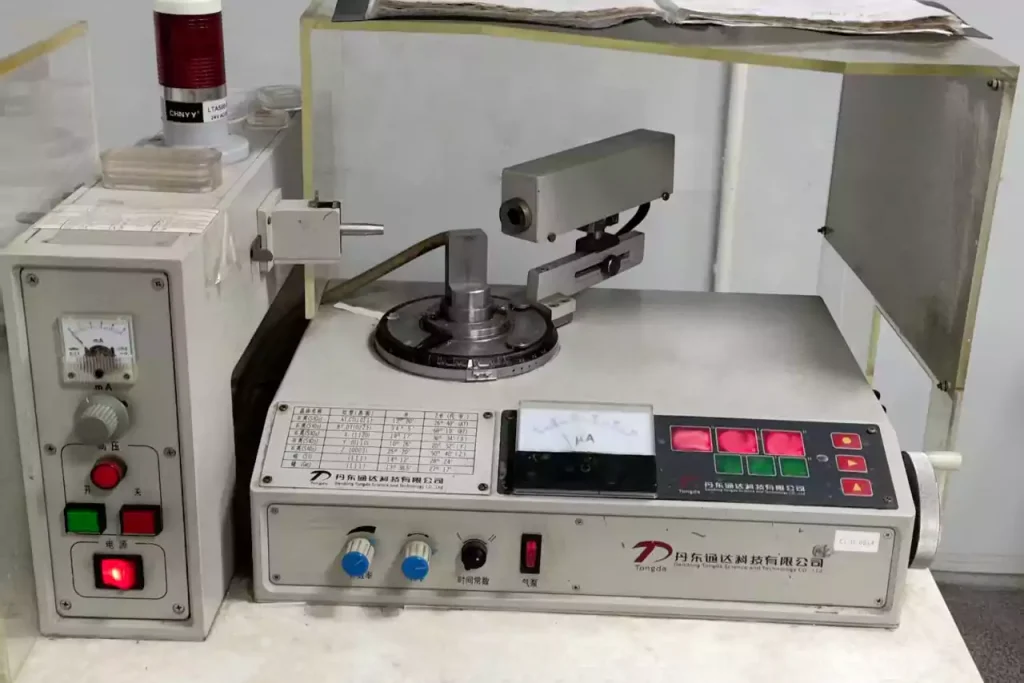
X ray Powder Diffractometer
Despite their advantages, optical methods have limitations, including stringent requirements for sample optical transparency. In practice, they are often combined with other techniques to compensate for these shortcomings. For example, when processing translucent crystals, integrating modern instrumentation can enhance accuracy and efficiency.
As a mature and user-friendly approach, optical methods remain irreplaceable in crystal orientation. Their simplicity and efficiency solidify their role as essential tools in both laboratory research and industrial production.
3.2 Mechanical Methods
Mechanical methods are traditional techniques that rely on direct observation of a crystal’s physical properties through cutting and polishing operations. These methods excel in preliminary orientation, particularly for scenarios requiring rapid crystallographic plane identification.
During mechanical orientation, operators typically expose a crystal’s internal structure by cutting its surface and then determine plane orientations using physical characteristics like light reflection or crack propagation. This approach is especially effective for initial orientation of large-sized crystals or industrial batch processing.
However, mechanical methods have limitations. Their accuracy is relatively low due to dependence on operator experience, and they are susceptible to environmental interference. For high-precision applications, mechanical techniques are often supplementary, combined with optical or modern instrumental methods.
Nevertheless, the low cost and operational simplicity of mechanical methods maintain their relevance in small-to-medium-scale crystal processing. Continuous improvements in cutting and polishing equipment have sustained the viability of this traditional approach in specific fields.
3.3 Modern Instrumentation Techniques
With technological advancements, modern instrumentation has become the mainstream tool for crystal orientation, with representative technologies including laser interferometers and electron microscopes. These techniques leverage precise optical or electronic principles to significantly enhance orientation accuracy and efficiency.
Laser interferometers utilize the interference properties of light waves to achieve sub-micron precision in measuring crystal surface topography. In crystal orientation, this technology is commonly used for positioning large or high-value crystals. For instance, in the manufacturing of optical window crystals, laser interferometers can rapidly assess surface flatness, providing critical reference data for subsequent processing.
Electron microscopes, on the other hand, employ high-energy electron beams to scan crystal surfaces or interiors, generating high-resolution images. This technique is particularly suited for analyzing micro-scale crystal structures, such as defect distributions or internal stress patterns. In modern materials science, electron microscopy has become indispensable for crystal orientation and structural analysis.
Compared to traditional methods, modern instrumentation offers superior precision and automation. However, these technologies come with higher costs and stringent operational requirements (e.g., cleanroom environments). Thus, they are typically reserved for high-end research or applications demanding extreme orientation accuracy.
The rapid development of modern instrumentation is propelling crystal orientation to new heights. Through integration with automation and artificial intelligence, these tools promise to further improve efficiency, unlocking new possibilities for scientific research and industrial production.
Chapter 4 Practical Applications of Crystal Orientation
4.1 Laser Industry
Crystal orientation plays a pivotal role in the laser industry, directly impacting laser performance and stability. For laser crystals like Nd:YAG and Ti:Sapphire, orientation accuracy determines output efficiency, beam quality, and operational reliability. For example, in Nd:YAG production, the [111] crystallographic direction is often selected as the optical axis to maximize gain performance and minimize transmission losses.
Moreover, crystal orientation mitigates thermal effects. Inaccurate orientation can induce thermal stress, leading to crystal cracking or beam distortion. Precise orientation optimizes thermal conductivity distribution, ensuring stable operation under high-power conditions.
As industrial demands grow, orientation requirements have become more stringent. Ultrafast lasers (e.g., femtosecond/picosecond lasers) require nanometer-level precision, achievable through advanced tools like laser interferometers and XRD, providing critical support for laser technology advancement.
4.2 Optical Device Manufacturing
Crystal orientation is equally vital in optical device fabrication. The performance of lenses, windows, and filters hinges on crystallographic alignment. For example, in high-performance optical windows, orientation dictates optical homogeneity and radiation resistance.
Specific crystallographic directions may exhibit lower birefringence or higher transmittance, directly influencing imaging quality and light transmission efficiency. In filter production, precise orientation prevents beam deviation, enhancing system accuracy.
Orientation also improves mechanical stability. For devices enduring high-intensity laser exposure, optimal orientation enhances thermal shock resistance and lifespan. Modern instrumentation accelerates orientation measurements, boosting production efficiency while reducing costs.
4.3 Materials Science
Crystal orientation is increasingly critical in materials science, particularly for functional and novel materials. Precise control over crystal structures unlocks latent properties, enabling breakthroughs in advanced technologies.
For example:
High-temperature superconductors: Oriented crystal growth enhances superconducting performance and conductivity, essential for energy storage devices.
Piezoelectric/photoelectric materials: Accurate orientation enables applications in sensors, acoustic devices, and optoelectronic converters.
Composite materials: Oriented assembly of crystals creates hybrids with multifunctional properties, such as optimized thermoelectric materials for renewable energy solutions.
In summary, crystal orientation is driving materials science toward unprecedented innovation, paving the way for next-generation technologies.
Chapter 5 Conclusion
Crystal orientation technology, as a vital branch of crystallography and materials science, permeates modern research and industrial applications. Its value is demonstrated across laser technology, optical manufacturing, and advanced materials development.
Theoretical foundations (e.g., crystal symmetry, Miller indices, Bragg’s Law) have not only underpinned orientation techniques but also enabled high-precision measurements. Methodologies span from optical/mechanical approaches for rapid assessment to cutting-edge instrumentation for nanometer-scale accuracy.
In practice, crystal orientation has elevated laser performance, refined optical device fabrication, and accelerated functional material innovation. These achievements highlight its versatility and potential to shape future scientific and industrial progress.
As automation and AI converge with orientation technologies, the field is poised to achieve even greater efficiency and precision, opening new frontiers in science and engineering.
For more articles, please visit our blog page
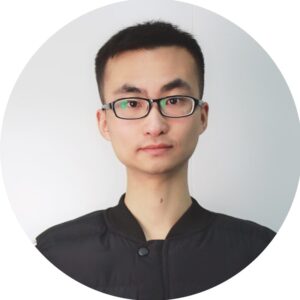
Frank
Frank graduated from the University of Shanghai for Science and Technology, majoring in optics. As a technical engineer at Crylink Company, he deeply understands crystal materials and laser components.
Related Video(s) with this Article
Related Product(s) with this Article
Related Application(s) with this Article